Fusion: Energy at the Extreme
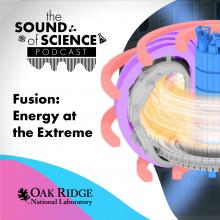
Building a sun on Earth to produce unlimited, carbon-free energy may sound like science fiction, but it's not. It's a nuclear process called fusion, where two atoms join together and create an abundance of energy. Recreating the power of a star is no easy feat, but scientists across the globe are hard at work to make it a reality. From materials, to confining sun-hot plasmas, to fuel, there are a lot of scientific challenges to overcome to build a fusion reactor. In this episode, we talked to several Oak Ridge National Laboratory scientists about how they are tackling these problems and why the future of fusion looks brighter than ever right now.
Transcript
[THEME MUSIC]
PHIL SNYDER: It could meet the energy needs of the whole planet for thousands of years, potentially.
CHARLES KESSEL: it makes you feel like you're really on the doorstep of something, because everybody's behind it now.
CAMI COLLINS: I truly believe that the future of our civilization depends on our ability to achieve fusion.
[MUSIC TRANSITION]
JENNY: Hello everyone and welcome to “The Sound of Science,” the podcast highlighting the voices behind the breakthroughs at Oak Ridge National Laboratory.
MORGAN/JENNY: We’re your hosts, Morgan McCorkle and Jenny Woodbery.
[MUSIC TRANSITION]
JENNY: In the south of France, a massive scientific experiment is under construction.
MORGAN: Nations from around the globe have come together to build one of the most ambitious energy projects in the world.
JENNY: Just what are they building? The sun – or something like it.
MORGAN: It’s a fusion device that will demonstrate the viability of producing power using the same nuclear processes that power the sun.
JENNY: The project is called ITER, which means “the way” in Latin. The results from this experiment could support a practical path to a new age of unlimited, carbon-free energy. Something desperately needed as climate change continues to progress.
MORGAN: But before we go any further, you may have a very simple question – what exactly is fusion?
CAMI COLLINS: Fusion is a process, it’s a nuclear process where two atoms join into one. It produces a lot of energy. It's the same process that the sun is powered by, all the stars. And it is something that we want to produce on Earth, because it can provide energy for thousands of years. The fuel is really abundant. We're talking about, on Earth, joining two hydrogen atoms to make helium.
JENNY: That’s Cami Collins. She’s a scientist and group leader in the advanced tokamak physics group fusion energy division at Oak Ridge. Don’t worry, we’ll tell you what a tokamak is a little later in the episode.
MORGAN: And while fusion may be new to some of us, Cami has been captivated by it since she was a kid.
COLLINS: I've actually been interested in fusion ever since fifth grade, when I very first learned that this is a process that doesn't produce greenhouse gases, has abundant fuel. It just clicked. I was like, why isn't everybody studying fusion? We should put all of our people into making this work.
JENNY: The promise of fusion has had scientists researching and developing ways to harness the power of the sun since the 1950s.
MORGAN: So why don’t we already have fusion energy? The answer to that is, well … complicated. As you can imagine, achieving fusion is no easy feat. We’re basically talking about re-creating the Sun on Earth. Remember the Sun is so large that more than a million Earths would fit inside of it. So it has some gravitational advantages when it comes to fusion.
JENNY: From materials, to confining sun-hot plasmas, to fuel, there are a lot of scientific challenges to overcome to build a fusion reactor. We talked to several ORNL scientists about how they are tackling these problems and why the future of fusion looks brighter than ever right now.
[MUSIC TRANSITION]
MORGAN: In the race to combat climate change, nuclear energy is a desirable energy source because it doesn’t produce carbon emissions or greenhouse gases.
JENNY: The U.S. has 56 operating nuclear power plants, but those reactors use a nuclear process called fission – not fusion.
MORGAN: And while they share some similarities, they’re very different processes—basically coming at reactions from different ends of the periodic table. Fission splits heavy elements. Fusion joins light elements, those with a small atomic number, like hydrogen.
COLLINS: The key difference is that fusion is joining, and fission is splitting. They're similar because they both they don't use fossil fuel. They both don't produce greenhouse gases. Fission requires special fuel like uranium, which is harder to get, and there's less of it. Actually the fuel for fusion is more plentiful, and we're talking about hydrogen that can be found from the ocean. It's not producing long-lived nuclear waste.
JENNY: The byproduct of a fusion reaction is helium – yes, the same element you use to inflate balloons for a birthday—plus a highly energetic neutron than can be useful for energy.
[MUSIC TRANSITON]
JENNY: As you’ve heard, the prospect of harnessing fusion is compelling for a number of reasons, but you may be wondering how it all works. So let’s break it down and meet some of the scientists working on fusion at the lab.
MORGAN: First, you need fuel. Unlike fission reactors, which rely on rare elements like uranium, the fuel for fusion is relatively easy to come by. The preferred fuel is made up of two forms, or isotopes, of hydrogen.
CHARLES KESSEL: One is called deuterium. And the other one is called tritium. Probably words you might not have heard of, very often. And deuterium is found mixed with normal water and just about anything else, that has hydrogen in it. Tritium, however, is does not occur naturally. And so, for us to use it as a fuel we have to make it and we do that by combining neutrons with lithium. So, there are nuclear reactions between neutrons and lithium that will produce tritium and so we rely on those reactions to make the fuel that we need there.
JENNY: Charles Kessel is a group leader in fusion nuclear science, technology and engineering at ORNL.
MORGAN: In order to make fusion happen, the fuel has to be extremely hot and confined within a defined space. Heating the hydrogen gas converts it into a plasma — a charged gas — which is a mixture of positive nuclei and negative electrons.
KESSEL: And the plasma is composed of deuterium and tritium. And in fact, the plasmas we use to make fusion energy are a million times less dense than the things around us in everyday life. So desks and air and cars and all the things around us. It’s very tenuous. And so in fact, we use very little amounts of material to generate the energy. And this is what's so attractive about nuclear energy, even fission is very similar, use very, very small amounts of material to generate very large amounts of energy.
JENNY: To control the plasma, it has to be confined in a fusion device with strong magnetic fields. One of the most common designs is called a tokamak. We asked Cami Collins to describe this unique device.
COLLINS: In order to get fusion to occur, we're talking about bringing two positive charges together, those are the nuclei. Positive charges don't want to go together. In order to get them close enough to overcome this electric repulsion, so a different force in nature takes over, the strong nuclear force, you have to get those nuclei very close together, which means they have to have a lot of energy. So they have to be hot. We have to create these hot temperatures on Earth in order to make fusion reactions. How do you do that? You can’t just capture it in any bottle you want, you need to have a special, special device that keeps such a hot material away from the walls. One concept is the tokamak, which is this magnetic confinement device. It’s in the shape of a doughnut, basically. You use this to capture the really hot plasma and keep it away from the walls of the device.
MORGAN: At ITER, scientists are assembling the biggest tokamak ever built – its magnetic doughnut is 10 stories tall.
JENNY: As you can imagine, the environment inside the tokamak is pretty extreme. The plasma in the core of the tokamak is far hotter than the core of the sun. Here’s Phil Snyder, the section head for burning plasma foundations at ORNL.
SNYDER: I mean, it just goes far beyond what we ever encounter in usual situations. And when those individual particles interact with materials, they are so energetic, that when they hit a material, they can knock atoms right off of the surface of the material, right, they don't just heat it up. You know, like, if you put, if you put a hot pan on your countertop, it'll heat the countertop. plasma is so hot that if each particle that hits your countertop would knock a particle off of your countertop, and it erode it away. And so we have to be clever about how how we cool down the plasma rapidly as it approaches the material surfaces and how we design the material surfaces.
MORGAN: While “holding” a plasma within a tokamak is primarily accomplished with magnetic fields, with such a harsh environment, you also need special materials that can withstand these crazy conditions.
JENNY: That’s what Lauren Garrison is focused on at Oak Ridge.
MORGAN: She’s a research and development staff member at the lab and the interim group leader for the advanced nuclear materials group in the Materials Science and Technology Division.
JENNY: A tokamak uses magnetic fields to suspend the super hot plasma off the inside of the device, and has several layers of materials on the plasma-facing surfaces. Lauren is studying the materials that can come in direct contact with the plasma.
LAUREN GARRISON: One measure of how harsh it is, is that for future devices and reactors in the future, we think we'll need to have that plasma facing material withstand probably, at least what we call 20 megawatts per meter squared of power, maybe up to 40 or higher. And so what that unit means -- that's the power coming onto that surface. And most people in our daily life, you never encounter megawatts per meter squared. Most people don't have a common sense about if that's high or low, or what that is. But one point of comparison, an estimate of the average heat flux on the surface of the sun is about 63 megawatts per meter squared. So when we're talking about needing something for 20, or 40 megawatts per meter squared, or maybe higher, we're in that kind of ballpark of approaching, you know, having a solid material right next to the sun. So it's quite harsh. And at the same time, those materials are withstanding both the heat flux and very high temperatures, they're being bombarded with neutrons, and they're also experiencing a big flux of ions coming that are exiting the plasma and hitting that front surface of the wall.
[MUSIC TRANSITION]
MORGAN: After decades of study, including a lot of new discoveries in the last ten years, scientists are closer than ever to achieving a path to fusion energy on a large scale.
JENNY Researchers have built hundreds of fusion devices – in fact you might have even heard of a high school student in Tennessee who built a small fusion reactor in his home. As incredible as that is, the problem with these devices is that they use more energy to create the fusion reactions than the power they produce.
MORGAN: That obviously, is an obstacle that needs to be overcome to make fusion energy practical – and one that ITER, the giant fusion experiment in France we mentioned earlier, is designed to address.
JENNY: The massive experiment will be the first to demonstrate a burning plasma - one that can continue heat itself once it is started up – and in doing so, produce more energy than it took to heat the plasma. A burning plasma can self-heat and can sustain the fusion reaction once the plasma’s core reaches around 200 million degrees. Phil Snyder says to think of it like a fire in your fireplace.
PHIL SNYDER: To get it started, you put a match in and that to begin with, you're putting heat into the process. But as the fire develops in and starts to burn significantly, it provides its own heat, and then it goes into a sustained state, where it continues to produce energy without your input. So, burning plasma is basically the same idea, except that the heating source is provided by the fusion reactions within the plasma.
MORGAN: But even as the world’s largest fusion device, ITER won’t be producing electricity for the power grid. ITER is an experimental facility. Researchers will use it to understand high power plasmas and gain real-world experience in operating a fusion reactor at a scale that’s never been tested before.
JENNY: There are still many questions, but the knowledge gained through ITER, along with other fusion research happening in parallel, will help scientists overcome the remaining challenges to making fusion energy a reality.
JENNY: Here’s Cami Collins again.
COLLINS: Generating electricity from fusion requires three main scientific and technological challenges to be met. The first one we have sort of the most experience with research, which is being able to control and sustain and predict this hot, high temperature, burning plasma. So we're working towards that. The second challenge, though, is actually to find materials that you can build your reactor out of. What can handle the extreme conditions of this fusion environment? And then the third challenge is being able to harness the fusion power. How do we capture the energy from the device? How do we keep the fuel cycle going?
[MUSIC TRANSITON]
MORGAN: Scientists at Oak Ridge are using the lab’s unique capabilities to tackle these challenges.
JENNY: To better understand and study the physics of burning plasmas, researchers are using modeling and simulation.
DAVID GREEN: As you can imagine, even if you had a little sun to work with, and you're trying to figure out how to build a reactor out of that, understanding what's happening inside there is probably pretty difficult to measure. You know, it's the sun, and it's very hot, dense, and it's not, it's not a place that's, well, it's an extreme environment, right. And while we have many smart people in fusion, designing very innovative diagnostics to try and measure what's happening inside a fusion plasma, it's impossible to know what's happening everywhere, at all times. So, you can sort of measure over here, over there. We’re certainly getting better at that. One of the things simulation allows you to do is know everything everywhere at all times within the limits of the assumptions of your model, and sort of how big your computer is.
MORGAN: That’s David Green. He’s the group leader for the plasma theory and modeling group within the fusion energy division at Oak Ridge.
JENNY: Luckily, ORNL has no shortage in computing power. The lab is home to Summit, the nation’s fastest supercomputer, and will soon welcome Frontier, anticipated to be one of the world’s first exascale systems.
MORGAN: Exascale computing systems at ORNL like Frontier will be capable of solving 1 quintillion calculations per second. That’s more than five times faster than today’s supercomputers.
JENNY: Even with extremely smart machines to work with, modeling plasma is a little tricky because the nature of a plasma is ... tricky.
MORGAN: We asked David to explain, but even the answer is a little complicated.
GREEN: I could give you a lot of math answers, but that's probably not going to be useful. The equations that describe their behavior are high dimensional. So typically, people think about anything about fluid flow, for example, like, or air flow around an airplane wing, you know, everybody's seen this idea of, you have an airplane wing and you see the fluid, the airflow around the wing, and then develop like turbulent structures going past. And this is like fluid dynamics, Navier-stokes equations, these sorts of things, and they're hard problems. And they have a lot of the same challenges as fusion in that there have lots of different scales. So either lots of little small details impact what the big picture is, but in fusion, instead of this problem just being a three dimensional problem, it's actually like a six dimensional problem. Because it depends not only on where you are in a plasma, but also where, how fast you're going, or where or where you came from in the past. And so the, the equations that underlie a plasma have inherently more complexity than those that you would typically use to describe a fluid, the type of fluid, the conventional fluids that people are used to. We’re trying to hold on to the plasma with a magnetic field, and that imposes a lot of extra physics in how the fluid behaves.
JENNY: All of the physics and math that goes into these models and simulations help scientists like Phil Snyder better predict the performance of the plasma -- leading to a better understanding of what is needed to achieve a self-sustaining fusion reaction.
SNYDER: It's really important from the point of view of eventually producing energy, producing electricity from fusion, tapping this enormous potential. I mean, essentially, fusion, because it produces so much energy from such a small amount of fuel that we can basically get from ocean water, it can produce, it can meet the energy needs of the whole planet for thousands of years, potentially. But in order to get to that state, we have to get the plasma conditions to where the fusion reactions can self-sustain. That the plasma can burn, so that we don't have to put in an enormous amount of energy in order to get out energy from the fusion reaction. So we have to get to the state where the plasma is producing a lot more energy via fusion than we're putting into it. And then we can extract that energy, run generators and produce clean electricity.
MORGAN: Modeling and simulation is also useful for other aspects of fusion as well. David and his colleagues are working towards creating integrated models that will help prepare for the design of a practical fusion power plant.
GREEN: We have lots of different types of models and simulation. And traditionally, a lot of them have been focused on how does the plasma behave when you, when you squeeze it with a more powerful magnetic field, or you heat it up? How does the plasma behave, but we're certainly looking to add more and more models, and I'm pushing in this direction towards outside of the plasma. And now that we understand the plasma to some level degree, like I said, there's more challenges to solve there. But moving outward towards the fuel cycle, and these other components of a reactor and trying to build or link all the models together, such that you can use them in this way to actually come up with a concept: what does a fusion pilot plant concept look like?
[MUSIC TRANSTION]
JENNY: Once a fusion reactor is up and running, even with a burning self-heated plasma, the reactions must be sustained over time by adding more fuel.
MORGAN: As you’ve heard, the fuel for fusion has two hydrogen isotopes – deuterium and tritium. Deuterium occurs naturally, but tritium has to be made.
JENNY: What’s cool about fusion – no pun intended – is the tritium can actually be produced as part of the fuel cycle.
MORGAN: We asked Charles Kessel to explain.
KESSEL: So what we have to do is we have to keep fueling it, we have to keep injecting more and more deuterium and tritium, because it's being consumed in these fusion reactions. And, and we also have to exhaust this fuel because it doesn't all burn. And so some of it comes back out, and we recover it, and we separate it, and then we send it back to fueling again. And this cycle goes around and around and around. And we need a certain amount of fuel to keep the plasma sustained and hot and burning. And we want it in a stationary state. We don’t want it going up and down, and have the power go up and down over and over – that’s very disruptive. Now we've consumed some of the fuel, which means we've consumed some of the tritium, which is something we can't find in nature. So we have to produce all the tritium that we burn. We have to produce more. And luckily, the deuterium-tritium fuel cycle, not only makes helium as a byproduct, but it also makes neutrons. And we can get those neutrons to combine with lithium by adding lithium materials around the plasma. And they will produce tritium. So we get the neutrons from the reaction to make the fuel that we actually just burned. And so we can close this fuel cycle in the fusion power plant. Now, when we make that tritium, we have to get it, we have to recover it, and we have to isolate it and purify it and so forth.
MORGAN: The way that the fuel is added is pretty unique. The deuterium and tritium are frozen and cut into tiny pellets that are injected into the burning plasma.
JENNY: Pellet injectors for fusion devices has been a specialty of ORNL’s for decades.
KESSEL: Oak Ridge supplies these pellet fueling systems to essentially every tokamak, every physics experiment around the world. And it’s going to have to get even better in order to use them in ITER and future nuclear devices. But what they have to do is, in fact, they have to obtain deuterium and tritium, they have to freeze it, they have to extrude it and turn it into ice and make it the right size, which turns out to be very challenging, but they've developed many very interesting techniques for doing it. Then they have to cut, turn it into little pellets, and then they fire it like a gun into the plasma. And this is the most effective way to deliver a lot of fuel in a small package into the plasma. Now, it's all about speed and position and so forth. And so a lot of work has gone into identifying how fast can I make these pellets go? And how can I aim my pellet gun so it goes in the right part of the plasma so it will be absorbed effectively. It's just fascinating.
MORGAN: Charles and his colleagues are not only studying what goes into the plasma, but also what comes out.
JENNY: Part of this has to do with the use of tritium. With a half-life of about 12 years, the radioactive material has to be securely managed onsite.
KESSEL: Many of the same experts who study pellet fueling are looking at what we do with the exhaust. And they're developing techniques to isolate the hydrogen and route it right back to fueling without any processing steps. And it turns out, this is very important, because it's a way to lower the total inventory of tritium at the plant. And the reason we like to do that is because tritium is radioactive. We don’t want it to be released. It’s vulnerable. So when it's sitting around, accidents can lead to it being released. And we don't want this to happen. So we want that inventory at the plant to be as small as it possibly can be. And so we're looking at methods to deal with the exhaust that let us get there.
[MUSIC TRANSITION]
MORGAN: The materials inside a fusion reactor have to withstand some of the most extreme environments in science. They face temperatures over 2,000 degrees Celsius and are constantly bombarded with energetic particles that threaten to change the structure of the material all together.
GARRISON: And one of the challenges is that the type of neutrons, the energy of neutrons that you have in fusion are much higher energy than we have in fission. So this is another challenge for us as material scientists is that it's a bit of a circular problem, you'd really like a fusion reactor, to test fusion materials to like expose them to neutrons, and then take them out and do tensile tests and hardness tests and break them in different ways and gather that data and use that data to build a fusion reactor. But of course, you can't do it in that order. So we have to make the best approximations we can and use all the other resources that are available.
JENNY: At ORNL, it’s Lauren Garrison’s job to investigate what kind of materials are best suited to face these harsh conditions.
MORGAN: One of the tools she uses to do this is the lab’s High Flux Isotope Reactor, or HFIR.
GARRISON: So really putting them through their paces, exposing them to high doses of neutrons in a test reactor, such as the High Flux Isotope Reactor, which is at Oak Ridge, and testing them to really make sure that they will last as long as we hope they will in fusion. So HFIR is a fission reactor. So it doesn't have quite the right energy of neutrons that we need. But it still is a fantastic tool for testing materials. And the fusion environment is more harsh than the fission environment, so at least we know if we irradiate it in HFIR, and if it looks good after that, then it's maybe a candidate that it could withstand the more harsh conditions in fusion. If we try something out, and we irradiate it in HFIR, and it falls apart, there's no way it's going to be any better in a fusion reactor. So HFIR is incredibly important for generating data to help our research directions with the materials.
JENNY: After the materials come out of HFIR, researchers examine them with an array of special techniques and tools.
GARRISON: We have our special laboratory where we are able to do tests of these neutron irradiated materials, which you can't just do anywhere, it takes special safety precautions and tools to be able to handle those materials in a safe way. So, we have unique microscopes for looking at the really small scale, at even down to the individual atom locations in materials. We have a whole bunch of mechanical testing equipment, which is very important for determining if the material is going to survive or not, we actually break it in many different ways to see how its response is. And that information tells us about then the limits of the material. So, if you know it breaks at a certain stress, then when you're designing your reactor, you'd better stay below that stress if you don't want the component to break.
MORGAN: Think of a tokamak like an onion. It has layers of materials leading to the core where the plasma is located. While the durability of all of the layers is important, it’s the plasma-facing material that has to survive the rigors of the fusion reaction.
JENNY: So what type of materials perform in that environment?
GARRISION: So one of the main materials that is the leading candidate is tungsten. And tungsten is actually just an element. So a simple element on the periodic table. And one of the reasons we like it for this application is that it has the highest melting temperature of any of the individual elements. And we know that this material is going to be at very high temperature and have to conduct out a lot of heat. But the problem with tungsten is it's extremely brittle. So what that means is, if there's a small crack, or if it's under a lot of stress, it really doesn't have much ability to bend or kind of move with the stress, it's much more likely that it would simply crack in half.
MORGAN: A brittle material is definitely not ideal for a practical fusion reactor that operates to deliver electricity every day, but Lauren and her colleagues are working on some creative ideas to improve this.
JENNY: One of those includes weaving together extremely fine strands of tungsten.
GARRISON: The easiest way to think about this is with concrete and steel rebar inside of it. So concrete is super brittle, it has a similar problem, and that if there's a crack, or it's under stress, it has a bad tendency to simply crack all the way in half. But if you take your concrete and you have bars of steel running through it, that adds a lot more strength and robustness to the whole system. So the idea is similar with tungsten, but on a really tiny scale. And instead of two different materials, we actually accomplish this all with tungsten.
MORGAN: Lauren’s worked with several iterations of this tungsten fiber. When they first started working with the fiber, it was about as thick as mechanical pencil lead. But now, the strands they’re working with are finer than a human hair.
GARRISON: I've been doing some experiments with 16 micron diameter tungsten fibers and these are so tiny, they are hard to see with your eye, you have to kind of get the light right to really see if it's there or not. If you have gloves on and you're trying to pick one up, you actually don't, or at least I don't have enough tactile sensation to tell if I'm holding it or not because it’s so tiny your fingers don’t even recognize it’s there. So this is very challenging on the experimental side. But once you make that into a composite, we think that will actually have benefits because whereas the small wires, they have some ability to flex, but not too much. But with the tiny, tiny fibers, you can actually use them in equipment that's used for weaving textiles, or weaving carbon fibers. So you can make tungsten fabric and tungsten weaves, which is just wild and fantastic, I think.
[MUSIC TRANSITION]
JENNY: Fusion is pretty mind-blowing. Creating energy from a star on earth almost sounds like something from science fiction.
MORGAN: But it’s real and it’s happening now. Construction at the ITER project is over 75 percent complete, and scientists are already working on the materials and technology for a fusion pilot plant that could lead us to practical fusion energy. As scientists get closer to realizing the potential of this game-changing energy source, there’s a tangible excitement in the field.
JENNY: Here’s Charles Kessel again.
KESSEL: As a community, a technical community, which is very broad, has a lot of different people with different disciplines, from plasma physics, to material science, to fusion engineering, even technology folks, everyone is agreeing, it's time to move out to fusion energy. This is a very rare consensus view. It makes you feel like you're really on the doorstep of something, because everybody's behind it now. And it just looks like we're ready to charge.
MORGAN: And in the face of the climate crisis, a carbon-free energy source like fusion, really can’t come soon enough. Here’s Cami Collins.
COLLINS: I truly believe that the future of our civilization depends on our ability to achieve fusion. We urgently need carbon free energy. If we want to reduce global warming, make sure that we have energy sources that are not polluting the Earth and causing temperatures to rise, we have to provide a lot of green energy sources to match the required energy capacity that's needed in the future. In the next 50 years, in the next 100 years, there's a huge projected need for just electricity in general, with world population growth, and we need to supply that with non-CO2 producing sources. Where is that going to come from? I think fusion is going to play a key role in that. So this is why it's, it's really important for us to get this working now.
[MUSIC TRANSITION]
JENNY: Thank you for listening to this episode of “The Sound of Science.” We hope you enjoyed it and will subscribe to the series wherever you get your podcasts.
MORGAN: If you want to learn more about this hot topic, we have a “Soundbite” coming out soon that highlights another fusion project the lab is working on – the Material Plasma Exposure eXperiment, also known as MPEX.
JENNY: And if you’re interested in tracking the progress of ITER, visit www.iter.org and www.usiter.org.
JENNY: Until next time!